A molecular switch controls assembly of bacterial focal adhesions
- PMID: 38809974
- PMCID: PMC11135422
- DOI: 10.1126/sciadv.adn2789
A molecular switch controls assembly of bacterial focal adhesions
Abstract
Cell motility universally relies on spatial regulation of focal adhesion complexes (FAs) connecting the substrate to cellular motors. In bacterial FAs, the Adventurous gliding motility machinery (Agl-Glt) assembles at the leading cell pole following a Mutual gliding-motility protein (MglA)-guanosine 5'-triphosphate (GTP) gradient along the cell axis. Here, we show that GltJ, a machinery membrane protein, contains cytosolic motifs binding MglA-GTP and AglZ and recruiting the MreB cytoskeleton to initiate movement toward the lagging cell pole. In addition, MglA-GTP binding triggers a conformational shift in an adjacent GltJ zinc-finger domain, facilitating MglB recruitment near the lagging pole. This prompts GTP hydrolysis by MglA, leading to complex disassembly. The GltJ switch thus serves as a sensor for the MglA-GTP gradient, controlling FA activity spatially.
Figures
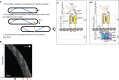
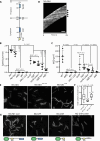
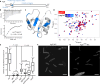
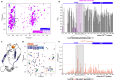
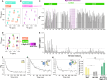
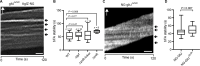
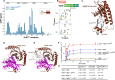
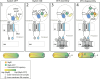
Similar articles
-
The small G-protein MglA connects to the MreB actin cytoskeleton at bacterial focal adhesions.J Cell Biol. 2015 Jul 20;210(2):243-56. doi: 10.1083/jcb.201412047. Epub 2015 Jul 13. J Cell Biol. 2015. PMID: 26169353 Free PMC article.
-
Spatial control of the GTPase MglA by localized RomR-RomX GEF and MglB GAP activities enables Myxococcus xanthus motility.Nat Microbiol. 2019 Aug;4(8):1344-1355. doi: 10.1038/s41564-019-0451-4. Epub 2019 May 20. Nat Microbiol. 2019. PMID: 31110363
-
A bacterial Ras-like small GTP-binding protein and its cognate GAP establish a dynamic spatial polarity axis to control directed motility.PLoS Biol. 2010 Jul 20;8(7):e1000430. doi: 10.1371/journal.pbio.1000430. PLoS Biol. 2010. PMID: 20652021 Free PMC article.
-
The mysterious nature of bacterial surface (gliding) motility: A focal adhesion-based mechanism in Myxococcus xanthus.Semin Cell Dev Biol. 2015 Oct;46:143-54. doi: 10.1016/j.semcdb.2015.10.033. Epub 2015 Oct 28. Semin Cell Dev Biol. 2015. PMID: 26520023 Review.
-
Myxococcus-from single-cell polarity to complex multicellular patterns.Annu Rev Genet. 2008;42:109-30. doi: 10.1146/annurev.genet.42.110807.091615. Annu Rev Genet. 2008. PMID: 18605899 Review.
Cited by
-
A miniTurbo-based proximity labeling protocol to identify conditional protein interactomes in vivo in Myxococcus xanthus.STAR Protoc. 2023 Dec 15;4(4):102657. doi: 10.1016/j.xpro.2023.102657. Epub 2023 Oct 25. STAR Protoc. 2023. PMID: 37883223 Free PMC article.
References
-
- Wozniak M. A., Modzelewska K., Kwong L., Keely P. J., Focal adhesion regulation of cell behavior. Biochim. Biophys. Acta 1692, 103–119 (2004). - PubMed
-
- Faure L. M., Fiche J.-B., Espinosa L., Ducret A., Anantharaman V., Luciano J., Lhospice S., Islam S. T., Tréguier J., Sotes M., Kuru E., Van Nieuwenhze M. S., Brun Y. V., Théodoly O., Aravind L., Nollmann M., Mignot T., The mechanism of force transmission at bacterial focal adhesion complexes. Nature 539, 530–535 (2016). - PMC - PubMed
MeSH terms
Substances
LinkOut - more resources
Full Text Sources
Research Materials
Miscellaneous