Gas and light: triggers of c-di-GMP-mediated regulation
- PMID: 37339911
- PMCID: PMC10505747
- DOI: 10.1093/femsre/fuad034
Gas and light: triggers of c-di-GMP-mediated regulation
Abstract
The widespread bacterial second messenger c-di-GMP is responsible for regulating many important physiological functions such as biofilm formation, motility, cell differentiation, and virulence. The synthesis and degradation of c-di-GMP in bacterial cells depend, respectively, on diguanylate cyclases and c-di-GMP-specific phosphodiesterases. Since c-di-GMP metabolic enzymes (CMEs) are often fused to sensory domains, their activities are likely controlled by environmental signals, thereby altering cellular c-di-GMP levels and regulating bacterial adaptive behaviors. Previous studies on c-di-GMP-mediated regulation mainly focused on downstream signaling pathways, including the identification of CMEs, cellular c-di-GMP receptors, and c-di-GMP-regulated processes. The mechanisms of CME regulation by upstream signaling modules received less attention, resulting in a limited understanding of the c-di-GMP regulatory networks. We review here the diversity of sensory domains related to bacterial CME regulation. We specifically discuss those domains that are capable of sensing gaseous or light signals and the mechanisms they use for regulating cellular c-di-GMP levels. It is hoped that this review would help refine the complete c-di-GMP regulatory networks and improve our understanding of bacterial behaviors in changing environments. In practical terms, this may eventually provide a way to control c-di-GMP-mediated bacterial biofilm formation and pathogenesis in general.
Keywords: NO sensors; c-di-GMP; c-di-GMP-specific phosphodiesterase; diguanylate cyclase; photoreceptors; sensory domains.
© The Author(s) 2023. Published by Oxford University Press on behalf of FEMS.
Conflict of interest statement
None declared.
Figures
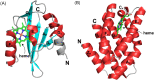
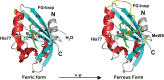
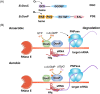
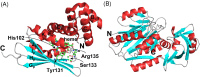
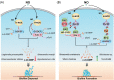
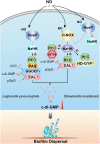
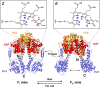
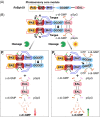
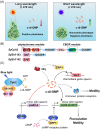
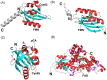
Similar articles
-
BolA Is Required for the Accurate Regulation of c-di-GMP, a Central Player in Biofilm Formation.mBio. 2017 Sep 19;8(5):e00443-17. doi: 10.1128/mBio.00443-17. mBio. 2017. PMID: 28928205 Free PMC article.
-
A c-di-GMP Signaling Cascade Controls Motility, Biofilm Formation, and Virulence in Burkholderia thailandensis.Appl Environ Microbiol. 2022 Apr 12;88(7):e0252921. doi: 10.1128/aem.02529-21. Epub 2022 Mar 24. Appl Environ Microbiol. 2022. PMID: 35323023 Free PMC article.
-
More than Enzymes That Make or Break Cyclic Di-GMP-Local Signaling in the Interactome of GGDEF/EAL Domain Proteins of Escherichia coli.mBio. 2017 Oct 10;8(5):e01639-17. doi: 10.1128/mBio.01639-17. mBio. 2017. PMID: 29018125 Free PMC article.
-
You've come a long way: c-di-GMP signaling.Curr Opin Microbiol. 2012 Apr;15(2):140-6. doi: 10.1016/j.mib.2011.12.008. Epub 2012 Jan 5. Curr Opin Microbiol. 2012. PMID: 22226607 Free PMC article. Review.
-
Sensory Perception in Bacterial Cyclic Diguanylate Signal Transduction.J Bacteriol. 2022 Feb 15;204(2):e0043321. doi: 10.1128/JB.00433-21. Epub 2021 Oct 4. J Bacteriol. 2022. PMID: 34606374 Free PMC article. Review.
Cited by
-
Stenotrophomonas maltophilia uses a c-di-GMP module to sense the mammalian body temperature during infection.PLoS Pathog. 2024 Sep 4;20(9):e1012533. doi: 10.1371/journal.ppat.1012533. eCollection 2024 Sep. PLoS Pathog. 2024. PMID: 39231185 Free PMC article.
-
Gene expression reprogramming of Pseudomonas alloputida in response to arginine through the transcriptional regulator ArgR.Microbiology (Reading). 2024 Mar;170(3):001449. doi: 10.1099/mic.0.001449. Microbiology (Reading). 2024. PMID: 38511653 Free PMC article.
-
Characterization of the dual regulation by a c-di-GMP riboswitch Bc1 with a long expression platform from Bacillus thuringiensis.Microbiol Spectr. 2024 Jul 2;12(7):e0045024. doi: 10.1128/spectrum.00450-24. Epub 2024 May 31. Microbiol Spectr. 2024. PMID: 38819160 Free PMC article.
-
Structures of the multi-domain oxygen sensor DosP: remote control of a c-di-GMP phosphodiesterase by a regulatory PAS domain.bioRxiv [Preprint]. 2024 Jul 24:2024.07.24.604967. doi: 10.1101/2024.07.24.604967. bioRxiv. 2024. Update in: Nat Commun. 2024 Nov 7;15(1):9653. doi: 10.1038/s41467-024-53942-7. PMID: 39091779 Free PMC article. Updated. Preprint.
-
Analyses of Xenorhabdus griffiniae genomes reveal two distinct sub-species that display intra-species variation due to prophages.BMC Genomics. 2024 Nov 15;25(1):1087. doi: 10.1186/s12864-024-10858-2. BMC Genomics. 2024. PMID: 39548374 Free PMC article.
References
-
- Abreu IA, Lourenço AI, Xavier AV et al. A novel iron centre in the split-soret cytochrome c from Desulfovibrio desulfuricans ATCC 27774. J Biol Inorg Chem. 2003;8:360–70. - PubMed
-
- Agari Y, Kashihara A, Yokoyama S et al. Global gene expression mediated by Thermus thermophilus SdrP, a CRP/FNR family transcriptional regulator. Mol Microbiol. 2008;70:60–75. - PubMed