Targeted polymeric therapeutic nanoparticles: design, development and clinical translation
- PMID: 22388185
- PMCID: PMC3684255
- DOI: 10.1039/c2cs15344k
Targeted polymeric therapeutic nanoparticles: design, development and clinical translation
Abstract
Polymeric materials have been used in a range of pharmaceutical and biotechnology products for more than 40 years. These materials have evolved from their earlier use as biodegradable products such as resorbable sutures, orthopaedic implants, macroscale and microscale drug delivery systems such as microparticles and wafers used as controlled drug release depots, to multifunctional nanoparticles (NPs) capable of targeting, and controlled release of therapeutic and diagnostic agents. These newer generations of targeted and controlled release polymeric NPs are now engineered to navigate the complex in vivo environment, and incorporate functionalities for achieving target specificity, control of drug concentration and exposure kinetics at the tissue, cell, and subcellular levels. Indeed this optimization of drug pharmacology as aided by careful design of multifunctional NPs can lead to improved drug safety and efficacy, and may be complimentary to drug enhancements that are traditionally achieved by medicinal chemistry. In this regard, polymeric NPs have the potential to result in a highly differentiated new class of therapeutics, distinct from the original active drugs used in their composition, and distinct from first generation NPs that largely facilitated drug formulation. A greater flexibility in the design of drug molecules themselves may also be facilitated following their incorporation into NPs, as drug properties (solubility, metabolism, plasma binding, biodistribution, target tissue accumulation) will no longer be constrained to the same extent by drug chemical composition, but also become in-part the function of the physicochemical properties of the NP. The combination of optimally designed drugs with optimally engineered polymeric NPs opens up the possibility of improved clinical outcomes that may not be achievable with the administration of drugs in their conventional form. In this critical review, we aim to provide insights into the design and development of targeted polymeric NPs and to highlight the challenges associated with the engineering of this novel class of therapeutics, including considerations of NP design optimization, development and biophysicochemical properties. Additionally, we highlight some recent examples from the literature, which demonstrate current trends and novel concepts in both the design and utility of targeted polymeric NPs (444 references).
Conflict of interest statement
The rest of the authors declare no conflict of interest.
Figures
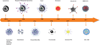
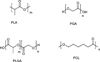
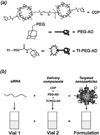
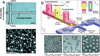
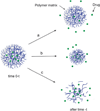
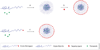
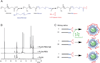
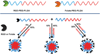
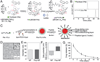
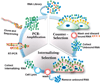
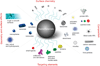
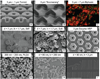
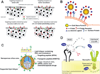
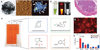
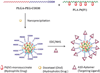
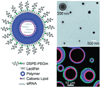
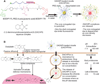
Similar articles
-
Self-assembled targeted nanoparticles: evolution of technologies and bench to bedside translation.Acc Chem Res. 2011 Oct 18;44(10):1123-34. doi: 10.1021/ar200054n. Epub 2011 Jun 21. Acc Chem Res. 2011. PMID: 21692448 Review.
-
Surface functionalization of polymeric nanoparticles for tumor drug delivery: approaches and challenges.Expert Opin Drug Deliv. 2017 Feb;14(2):201-214. doi: 10.1080/17425247.2016.1213238. Epub 2016 Jul 27. Expert Opin Drug Deliv. 2017. PMID: 27426638 Review.
-
Theranostic Nanoparticles for RNA-Based Cancer Treatment.Acc Chem Res. 2019 Jun 18;52(6):1496-1506. doi: 10.1021/acs.accounts.9b00101. Epub 2019 May 28. Acc Chem Res. 2019. PMID: 31135134 Free PMC article. Review.
-
Biodegradable polymeric nanoparticles administered in the cerebrospinal fluid: Brain biodistribution, preferential internalization in microglia and implications for cell-selective drug release.Biomaterials. 2019 Jul;209:25-40. doi: 10.1016/j.biomaterials.2019.04.012. Epub 2019 Apr 14. Biomaterials. 2019. PMID: 31026609
-
Polymeric nanoparticles as carrier for targeted and controlled delivery of anticancer agents.Ther Deliv. 2019 Aug;10(8):527-550. doi: 10.4155/tde-2019-0044. Epub 2019 Sep 9. Ther Deliv. 2019. PMID: 31496433 Review.
Cited by
-
Cancer nanomedicines: closing the translational gap.Lancet. 2014 Dec 20;384(9961):2175-6. doi: 10.1016/S0140-6736(14)61457-4. Epub 2014 Dec 19. Lancet. 2014. PMID: 25625382 Free PMC article. No abstract available.
-
Complete Regression of Xenograft Tumors upon Targeted Delivery of Paclitaxel via Π-Π Stacking Stabilized Polymeric Micelles.ACS Nano. 2015;9(4):3740-52. doi: 10.1021/acsnano.5b00929. Epub 2015 Apr 6. ACS Nano. 2015. PMID: 25831471 Free PMC article.
-
Multifunctional Polymeric Micelles for Cancer Therapy.Polymers (Basel). 2022 Nov 10;14(22):4839. doi: 10.3390/polym14224839. Polymers (Basel). 2022. PMID: 36432965 Free PMC article. Review.
-
TPGS Decorated Liposomes as Multifunctional Nano-Delivery Systems.Pharm Res. 2023 Jan;40(1):245-263. doi: 10.1007/s11095-022-03424-6. Epub 2022 Nov 14. Pharm Res. 2023. PMID: 36376604 Free PMC article. Review.
-
Challenges and key considerations of the enhanced permeability and retention effect for nanomedicine drug delivery in oncology.Cancer Res. 2013 Apr 15;73(8):2412-7. doi: 10.1158/0008-5472.CAN-12-4561. Epub 2013 Feb 19. Cancer Res. 2013. PMID: 23423979 Free PMC article.
References
-
- LaVan DA, McGuire T, Langer R. Nat. Biotechnol. 2003;21:1184–1191. - PubMed
-
- Langer R. Science. 1990;249:1527–1533. - PubMed
-
- Shi J, Xiao Z, Kamaly N, Farokhzad OC. Acc. Chem. Res. 2011;44:1123–1134. - PubMed
-
- Davis ME, Chen ZG, Shin DM. Nat. Rev. Drug Discovery. 2008;7:771–782. - PubMed
-
- Farokhzad OC. Expert Opin. Drug Delivery. 2008;5:927–929. - PubMed
Publication types
MeSH terms
Substances
Grants and funding
LinkOut - more resources
Full Text Sources
Other Literature Sources
Miscellaneous