Stimulus-responsive self-assembled prodrugs in cancer therapy
- PMID: 35509461
- PMCID: PMC9006903
- DOI: 10.1039/d2sc01003h
Stimulus-responsive self-assembled prodrugs in cancer therapy
Abstract
Small-molecule prodrugs have become the main toolbox to improve the unfavorable physicochemical properties of potential therapeutic compounds in contemporary anti-cancer drug development. Many approved small-molecule prodrugs, however, still face key challenges in their pharmacokinetic (PK) and pharmacodynamic (PD) properties, thus severely restricting their further clinical applications. Self-assembled prodrugs thus emerged as they could take advantage of key benefits in both prodrug design and nanomedicine, so as to maximize drug loading, reduce premature leakage, and improve PK/PD parameters and targeting ability. Notably, temporally and spatially controlled release of drugs at cancerous sites could be achieved by encoding various activable linkers that are sensitive to chemical or biological stimuli in the tumor microenvironment (TME). In this review, we have comprehensively summarized the recent progress made in the development of single/multiple-stimulus-responsive self-assembled prodrugs for mono- and combinatorial therapy. A special focus was placed on various prodrug conjugation strategies (polymer-drug conjugates, drug-drug conjugates, etc.) that facilitated the engineering of self-assembled prodrugs, and various linker chemistries that enabled selective controlled release of active drugs at tumor sites. Furthermore, some polymeric nano-prodrugs that entered clinical trials have also been elaborated here. Finally, we have discussed the bottlenecks in the field of prodrug nanoassembly and offered potential solutions to overcome them. We believe that this review will provide a comprehensive reference for the rational design of effective prodrug nanoassemblies that have clinic translation potential.
This journal is © The Royal Society of Chemistry.
Conflict of interest statement
The authors declare no competing financial interest.
Figures
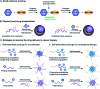
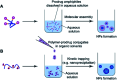
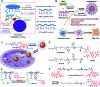
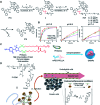
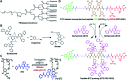
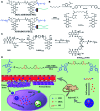
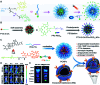
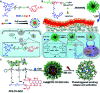
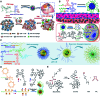
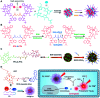
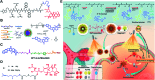
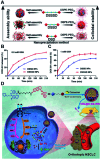
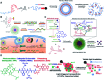
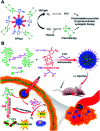
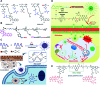
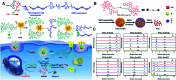
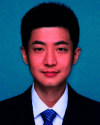
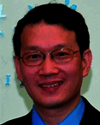
Similar articles
-
Dimeric prodrug-based nanomedicines for cancer therapy.J Control Release. 2020 Oct 10;326:510-522. doi: 10.1016/j.jconrel.2020.07.036. Epub 2020 Jul 25. J Control Release. 2020. PMID: 32721523 Review.
-
Stimuli-Responsive Prodrug Chemistries for Cancer Therapy.Chembiochem. 2023 Sep 15;24(18):e202300155. doi: 10.1002/cbic.202300155. Epub 2023 Aug 18. Chembiochem. 2023. PMID: 37341379 Review.
-
Stimuli-responsive prodrug-based cancer nanomedicine.EBioMedicine. 2020 Jun;56:102821. doi: 10.1016/j.ebiom.2020.102821. Epub 2020 Jun 7. EBioMedicine. 2020. PMID: 32505922 Free PMC article. Review.
-
Prodrug-based nanoparticulate drug delivery strategies for cancer therapy.Trends Pharmacol Sci. 2014 Nov;35(11):556-66. doi: 10.1016/j.tips.2014.09.008. Epub 2014 Oct 16. Trends Pharmacol Sci. 2014. PMID: 25441774 Review.
-
Recent trends in bioresponsive linker technologies of Prodrug-Based Self-Assembling nanomaterials.Biomaterials. 2021 Aug;275:120955. doi: 10.1016/j.biomaterials.2021.120955. Epub 2021 Jun 5. Biomaterials. 2021. PMID: 34130143 Review.
Cited by
-
pH-Responsive nanofiber buttresses as local drug delivery devices.Biomater Sci. 2023 Jan 31;11(3):813-821. doi: 10.1039/d2bm01199a. Biomater Sci. 2023. PMID: 36408890 Free PMC article.
-
Peptide nano 'bead-grafting' for SDT-facilitated immune checkpoints blocking.Chem Sci. 2022 Nov 23;13(47):14052-14062. doi: 10.1039/d2sc02728c. eCollection 2022 Dec 7. Chem Sci. 2022. PMID: 36540822 Free PMC article.
-
Cooperatively enhanced photothermal-chemotherapy via simultaneously downregulating HSPs and promoting DNA alkylation in cancer cells.Chem Sci. 2022 Dec 28;14(4):1010-1017. doi: 10.1039/d2sc06143k. eCollection 2023 Jan 25. Chem Sci. 2022. PMID: 36755714 Free PMC article.
-
Dynamic crosslinked polymeric nano-prodrugs for highly selective synergistic chemotherapy.Asian J Pharm Sci. 2022 Nov;17(6):880-891. doi: 10.1016/j.ajps.2022.09.004. Epub 2022 Oct 29. Asian J Pharm Sci. 2022. PMID: 36600901 Free PMC article.
-
Smart Delivery Systems Responsive to Cathepsin B Activity for Cancer Treatment.Pharmaceutics. 2023 Jun 29;15(7):1848. doi: 10.3390/pharmaceutics15071848. Pharmaceutics. 2023. PMID: 37514035 Free PMC article. Review.
References
-
- Markham M. J. Wachter K. Agarwal N. Bertagnolli M. M. Chang S. M. Dale W. Diefenbach C. S. M. Rodriguez-Galindo C. George D. J. Gilligan T. D. Harvey R. D. Johnson M. L. Kimple R. J. Knoll M. A. LoConte N. Maki R. G. Meisel J. L. Meyerhardt J. A. Pennell N. A. Rocque G. B. Sabel M. S. Schilsky R. L. Schneider B. J. Tap W. D. Uzzo R. G. Westin S. N. J. Clin. Oncol. 2020;38:1081. doi: 10.1200/JCO.19.03141. - DOI - PubMed
Publication types
LinkOut - more resources
Full Text Sources