Vitamin C: the known and the unknown and Goldilocks
- PMID: 26808119
- PMCID: PMC4959991
- DOI: 10.1111/odi.12446
Vitamin C: the known and the unknown and Goldilocks
Abstract
Vitamin C (Ascorbic Acid), the antiscorbutic vitamin, cannot be synthesized by humans and other primates, and has to be obtained from diet. Ascorbic acid is an electron donor and acts as a cofactor for fifteen mammalian enzymes. Two sodium-dependent transporters are specific for ascorbic acid, and its oxidation product dehydroascorbic acid is transported by glucose transporters. Ascorbic acid is differentially accumulated by most tissues and body fluids. Plasma and tissue vitamin C concentrations are dependent on amount consumed, bioavailability, renal excretion, and utilization. To be biologically meaningful or to be clinically relevant, in vitro and in vivo studies of vitamin C actions have to take into account physiologic concentrations of the vitamin. In this paper, we review vitamin C physiology; the many phenomena involving vitamin C where new knowledge has accrued or where understanding remains limited; raise questions about the vitamin that remain to be answered; and explore lines of investigations that are likely to be fruitful.
Keywords: dehydroascorbic acid; dose-concentration relationship; recommended dietary allowance; scurvy; vitamin C; vitamin C transport.
Published 2016. This article is a U.S. Government work and is in the public domain in the USA.
Figures
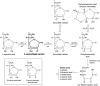
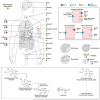
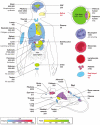
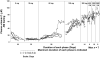
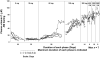
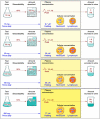
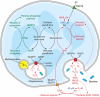
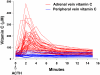
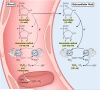
Similar articles
-
Colony-stimulating factors signal for increased transport of vitamin C in human host defense cells.Blood. 1998 Apr 1;91(7):2536-46. Blood. 1998. PMID: 9516155
-
Increased facilitated transport of dehydroascorbic acid without changes in sodium-dependent ascorbate transport in human melanoma cells.Cancer Res. 1997 Jun 15;57(12):2529-37. Cancer Res. 1997. PMID: 9192836
-
Recycling of vitamin C by a bystander effect.J Biol Chem. 2003 Mar 21;278(12):10128-33. doi: 10.1074/jbc.M210686200. Epub 2002 Nov 14. J Biol Chem. 2003. PMID: 12435736
-
Vitamin C and the role of citrus juices as functional food.Nat Prod Commun. 2009 May;4(5):677-700. Nat Prod Commun. 2009. PMID: 19445318 Review.
-
Vitamin C transporters.J Physiol Biochem. 2008 Dec;64(4):357-75. doi: 10.1007/BF03174092. J Physiol Biochem. 2008. PMID: 19391462 Review.
Cited by
-
Dynamic changes in genomic 5-hydroxymethyluracil and N6-methyladenine levels in the Drosophila melanogaster life cycle and in response to different temperature conditions.Sci Rep. 2022 Oct 20;12(1):17552. doi: 10.1038/s41598-022-22490-9. Sci Rep. 2022. PMID: 36266436 Free PMC article.
-
Iron metabolism mediates the relationship between Vitamin C and hepatic steatosis and fibrosis in NAFLD.Front Nutr. 2022 Sep 8;9:952056. doi: 10.3389/fnut.2022.952056. eCollection 2022. Front Nutr. 2022. PMID: 36159474 Free PMC article.
-
Maternal Vitamin C and Iron Intake during Pregnancy and the Risk of Islet Autoimmunity and Type 1 Diabetes in Children: A Birth Cohort Study.Nutrients. 2021 Mar 13;13(3):928. doi: 10.3390/nu13030928. Nutrients. 2021. PMID: 33805588 Free PMC article.
-
Hypertrophic cardiomyopathy-associated mutations drive stromal activation via EGFR-mediated paracrine signaling.Sci Adv. 2024 Oct 18;10(42):eadi6927. doi: 10.1126/sciadv.adi6927. Epub 2024 Oct 16. Sci Adv. 2024. PMID: 39413182 Free PMC article.
-
The Oxidative Drug Combination for Suppressing KRAS G12D Inducible Tumour Growth.Biomed Res Int. 2022 Dec 30;2022:9426623. doi: 10.1155/2022/9426623. eCollection 2022. Biomed Res Int. 2022. PMID: 36619305 Free PMC article.
References
-
- Amir Shaghaghi M, Bernstein CN, Serrano Leon A, El-Gabalawy H, Eck P. Polymorphisms in the sodium-dependent ascorbate transporter gene SLC23A1 are associated with susceptibility to Crohn disease. Am J Clin Nutr. 2014;99:378–83. - PubMed
-
- Anderson R, Lukey PT. A biological role for ascorbate in the selective neutralization of extracellular phagocyte-derived oxidants. Ann N Y Acad Sci. 1987;498:229–47. - PubMed
-
- Anonymous Ascorbic acid intake and salivary ascorbate levels. Nutr Rev. 1986;44:328–30. - PubMed
Publication types
MeSH terms
Substances
Grants and funding
LinkOut - more resources
Full Text Sources
Other Literature Sources
Medical