Mechanism of fatty-acid-dependent UCP1 uncoupling in brown fat mitochondria
- PMID: 23063128
- PMCID: PMC3782081
- DOI: 10.1016/j.cell.2012.09.010
Mechanism of fatty-acid-dependent UCP1 uncoupling in brown fat mitochondria
Abstract
Mitochondrial uncoupling protein 1 (UCP1) is responsible for nonshivering thermogenesis in brown adipose tissue (BAT). Upon activation by long-chain fatty acids (LCFAs), UCP1 increases the conductance of the inner mitochondrial membrane (IMM) to make BAT mitochondria generate heat rather than ATP. Despite being a member of the family of mitochondrial anion carriers (SLC25), UCP1 is believed to transport H(+) by an unusual mechanism that has long remained unresolved. Here, we achieved direct patch-clamp measurements of UCP1 currents from the IMM of BAT mitochondria. We show that UCP1 is an LCFA anion/H(+) symporter. However, the LCFA anions cannot dissociate from UCP1 due to hydrophobic interactions established by their hydrophobic tails, and UCP1 effectively operates as an H(+) carrier activated by LCFA. A similar LCFA-dependent mechanism of transmembrane H(+) transport may be employed by other SLC25 members and be responsible for mitochondrial uncoupling and regulation of metabolic efficiency in various tissues.
Copyright © 2012 Elsevier Inc. All rights reserved.
Figures
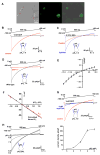
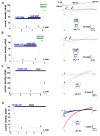
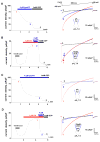
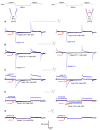
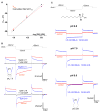
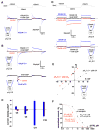
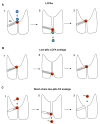
Similar articles
-
UCP1: A transporter for H+ and fatty acid anions.Biochimie. 2017 Mar;134:28-34. doi: 10.1016/j.biochi.2016.10.013. Epub 2016 Oct 27. Biochimie. 2017. PMID: 27984203 Free PMC article. Review.
-
The Mechanism FA-Dependent H+ Transport by UCP1.Handb Exp Pharmacol. 2019;251:143-159. doi: 10.1007/164_2018_138. Handb Exp Pharmacol. 2019. PMID: 29797089
-
Uncoupling protein 1 decreases superoxide production in brown adipose tissue mitochondria.J Biol Chem. 2010 Jul 16;285(29):21961-8. doi: 10.1074/jbc.M110.122861. Epub 2010 May 13. J Biol Chem. 2010. PMID: 20466728 Free PMC article.
-
Within brown-fat cells, UCP1-mediated fatty acid-induced uncoupling is independent of fatty acid metabolism.Biochim Biophys Acta. 2008 Jul-Aug;1777(7-8):642-50. doi: 10.1016/j.bbabio.2008.04.038. Epub 2008 May 1. Biochim Biophys Acta. 2008. PMID: 18489899
-
The molecular features of uncoupling protein 1 support a conventional mitochondrial carrier-like mechanism.Biochimie. 2017 Mar;134:35-50. doi: 10.1016/j.biochi.2016.12.016. Epub 2017 Jan 3. Biochimie. 2017. PMID: 28057583 Free PMC article. Review.
Cited by
-
Selective Kv1.3 channel blocker as therapeutic for obesity and insulin resistance.Proc Natl Acad Sci U S A. 2013 Jun 11;110(24):E2239-48. doi: 10.1073/pnas.1221206110. Epub 2013 May 31. Proc Natl Acad Sci U S A. 2013. PMID: 23729813 Free PMC article.
-
Testosterone treatment is associated with reduced adipose tissue dysfunction and nonalcoholic fatty liver disease in obese hypogonadal men.J Endocrinol Invest. 2021 Apr;44(4):819-842. doi: 10.1007/s40618-020-01381-8. Epub 2020 Aug 8. J Endocrinol Invest. 2021. PMID: 32772323 Free PMC article.
-
Skeletal integration of energy homeostasis: Translational implications.Bone. 2016 Jan;82:35-41. doi: 10.1016/j.bone.2015.07.026. Epub 2015 Jul 23. Bone. 2016. PMID: 26211994 Free PMC article. Review.
-
Metabolically inert perfluorinated fatty acids directly activate uncoupling protein 1 in brown-fat mitochondria.Arch Toxicol. 2016 May;90(5):1117-28. doi: 10.1007/s00204-015-1535-4. Epub 2015 Jun 4. Arch Toxicol. 2016. PMID: 26041126 Free PMC article.
-
Fatty acid metabolism and the basis of brown adipose tissue function.Adipocyte. 2015 Dec 10;5(2):98-118. doi: 10.1080/21623945.2015.1122857. eCollection 2016 Apr-Jun. Adipocyte. 2015. PMID: 27386151 Free PMC article. Review.
References
-
- Anel A, Richieri GV, Kleinfeld AM. Membrane partition of fatty acids and inhibition of T cell function. Biochemistry. 1993;32:530–536. - PubMed
-
- Annunziata O, Costantino L, D’Errico G, Paduano L, Vitagliano VV. Transport Properties for Aqueous Sodium Sulfonate Surfactants. J Colloid Interface Sci. 1999;216:16–24. - PubMed
-
- Bezanilla F. The voltage sensor in voltage-dependent ion channels. Physiol Rev. 2000;80:555–592. - PubMed
-
- Bhamidipati SP, Hamilton JA. Interactions of lyso 1-palmitoylphosphatidylcholine with phospholipids: a 13C and 31P NMR study. Biochemistry. 1995;34:5666–5677. - PubMed
Publication types
MeSH terms
Substances
Grants and funding
LinkOut - more resources
Full Text Sources
Other Literature Sources
Molecular Biology Databases
Research Materials