Nonfunctional coq10 mutants maintain the ERMES complex and reveal true phenotypes associated with the loss of the coenzyme Q chaperone protein Coq10
- PMID: 39343004
- PMCID: PMC11541779
- DOI: 10.1016/j.jbc.2024.107820
Nonfunctional coq10 mutants maintain the ERMES complex and reveal true phenotypes associated with the loss of the coenzyme Q chaperone protein Coq10
Abstract
Coenzyme Q (CoQ) is a redox-active lipid molecule that acts as an electron carrier in the mitochondrial electron transport chain. In Saccharomyces cerevisiae, CoQ is synthesized in the mitochondrial matrix by a multisubunit protein-lipid complex termed the CoQ synthome, the spatial positioning of which is coordinated by the endoplasmic reticulum-mitochondria encounter structure (ERMES). The MDM12 gene encoding the cytosolic subunit of ERMES is coexpressed with COQ10, which encodes the putative CoQ chaperone Coq10, via a shared bidirectional promoter. Deletion of COQ10 results in respiratory deficiency, impaired CoQ biosynthesis, and reduced spatial coordination between ERMES and the CoQ synthome. While Coq10 protein content is maintained upon deletion of MDM12, we show that deletion of COQ10 by replacement with a HIS3 marker results in diminished Mdm12 protein content. Since deletion of individual ERMES subunits prevents ERMES formation, we asked whether some or all of the phenotypes associated with COQ10 deletion result from ERMES dysfunction. To identify the phenotypes resulting solely due to the loss of Coq10, we constructed strains expressing a functionally impaired (coq10-L96S) or truncated (coq10-R147∗) Coq10 isoform using CRISPR-Cas9. We show that both coq10 mutants preserve Mdm12 protein content and exhibit impaired respiratory capacity like the coq10Δ mutant, indicating that Coq10's function is vital for respiration regardless of ERMES integrity. Moreover, the maintenance of CoQ synthome stability and efficient CoQ biosynthesis observed for the coq10-R147∗ mutant suggests these deleterious phenotypes in the coq10Δ mutant result from ERMES disruption. Overall, this study clarifies the role of Coq10 in modulating CoQ biosynthesis.
Keywords: CoQ synthome; Coq10; Mdm12; START domain; Saccharomyces cerevisiae; coenzyme Q; endoplasmic reticulum-mitochondria encounter structure; lipid; mitochondrial metabolism; ubiquinone.
Copyright © 2024 The Authors. Published by Elsevier Inc. All rights reserved.
Conflict of interest statement
Conflict of interest The authors declare that they have no conflicts of interest with the contents of this article.
Figures
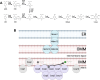
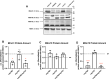
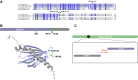
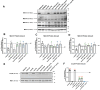
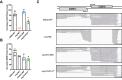
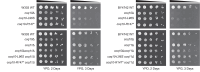
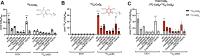
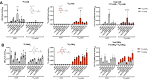
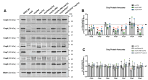
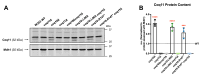
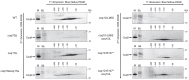
Similar articles
-
Mitochondrial-ER Contact Sites and Tethers Influence the Biosynthesis and Function of Coenzyme Q.Contact (Thousand Oaks). 2025 Feb 3;8:25152564251316350. doi: 10.1177/25152564251316350. eCollection 2025 Jan-Dec. Contact (Thousand Oaks). 2025. PMID: 39906518 Free PMC article.
-
Depressing time: Waiting, melancholia, and the psychoanalytic practice of care.In: Kirtsoglou E, Simpson B, editors. The Time of Anthropology: Studies of Contemporary Chronopolitics. Abingdon: Routledge; 2020. Chapter 5. In: Kirtsoglou E, Simpson B, editors. The Time of Anthropology: Studies of Contemporary Chronopolitics. Abingdon: Routledge; 2020. Chapter 5. PMID: 36137063 Free Books & Documents. Review.
-
Histology, Axon.2022 Nov 14. In: StatPearls [Internet]. Treasure Island (FL): StatPearls Publishing; 2025 Jan–. 2022 Nov 14. In: StatPearls [Internet]. Treasure Island (FL): StatPearls Publishing; 2025 Jan–. PMID: 32119275 Free Books & Documents.
-
COQ11 deletion mitigates respiratory deficiency caused by mutations in the gene encoding the coenzyme Q chaperone protein Coq10.J Biol Chem. 2020 May 1;295(18):6023-6042. doi: 10.1074/jbc.RA119.012420. Epub 2020 Mar 23. J Biol Chem. 2020. PMID: 32205446 Free PMC article.
-
Antioxidants for female subfertility.Cochrane Database Syst Rev. 2017 Jul 28;7(7):CD007807. doi: 10.1002/14651858.CD007807.pub3. Cochrane Database Syst Rev. 2017. Update in: Cochrane Database Syst Rev. 2020 Aug 27;8:CD007807. doi: 10.1002/14651858.CD007807.pub4. PMID: 28752910 Free PMC article. Updated. Review.
References
-
- Banerjee R., Purhonen J., Kallijarvi J. The mitochondrial coenzyme Q junction and complex III: biochemistry and pathophysiology. FEBS J. 2022;289:6936–6958. - PubMed
-
- Okada K., Suzuki K., Kamiya Y., Zhu X., Fujisaki S., Nishimura Y., et al. Polyprenyl diphosphate synthase essentially defines the length of the side chain of ubiquinone. Biochim. Biophys. Acta. 1996;1302:217–223. - PubMed